Table of Content
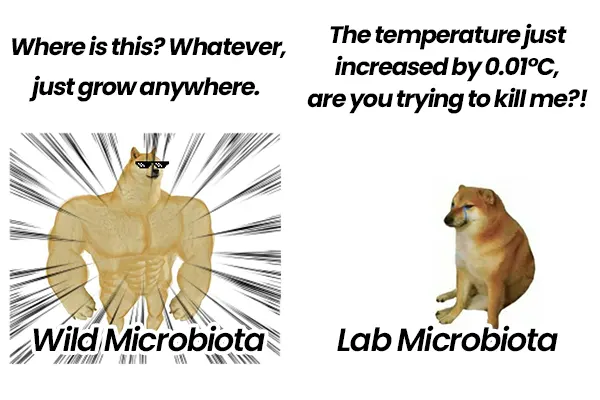
The Complexity of Natural Microbial Communities
Microbial Diversity
Microbial Diversity
Microbial Interactions
Microbial Interactions

Challenges in Laboratory Replication
Challenges in Laboratory Replication
Limitations of Growth Media
Nutrient Composition
Nutrient Composition
Static Conditions vs. Dynamic Nutrients
Static Conditions vs. Dynamic Nutrients
Environmental Control Challenges
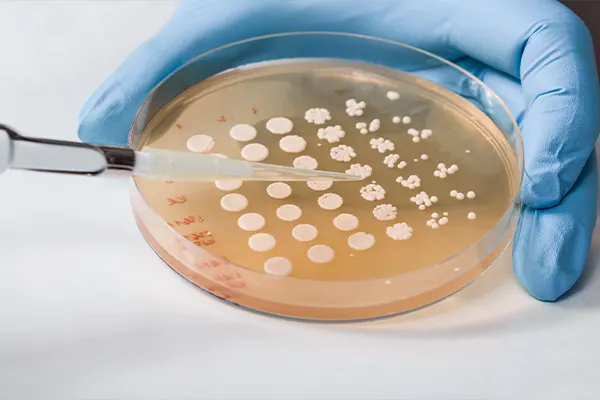
Oxygen Gradients
Temperature and pH Dynamics
In conclusion, while laboratory control of environmental factors such as oxygen, temperature, and pH is essential for studying bacteria, these static parameters fall short of replicating the dynamic and heterogeneous conditions of natural ecosystems. The inability to mimic such variations limits the cultivation and understanding of many bacterial species, particularly those adapted to fluctuating environments. Recognizing these limitations is a critical step toward developing more sophisticated cultivation techniques that better capture the complexity of natural microbial habitats.
Emerging Technologies and Solutions
Co-Culture Systems
Co-Culture Systems
Microfluidic Technology
Microfluidic Technology

Metagenomics and Synthetic Communities
Metagenomics and Synthetic Communities
Conclusion
Growing perfect bacterial flora in the lab is challenging due to the immense complexity of natural microbial communities, limitations in traditional growth media, and the difficulty of replicating dynamic environmental conditions. However, emerging technologies like co-culture systems, microfluidics, and metagenomics offer promising solutions to overcome these barriers.
By improving our ability to cultivate diverse bacterial species and simulate natural ecosystems, researchers can unlock valuable insights into microbial ecology, human health, and environmental science. The pursuit of perfect bacterial flora may remain difficult, but ongoing innovations are bringing us closer to this goal.
Recent Posts
How to rapidly detect Monkeypox by real-time PCR
Based on the full sequence of monkeypox virus (AF380138) F3L gene published in GenBank, primers, TaqMan and MGB probes were designed and synthesized, and a fluorescent quantitative PCR detection method for F3L gene was established. [...]
For beginners: 16 professional terms and common questions you feel confused about Real-time PCR
When you just graduated and entered the job, came into contact with the real-time quantitative PCR experiment, faced with a bunch of nouns, are you confused about where to start? What is the [...]
How to choose consumables for experiment: 8 different aspects of PCR consumables you need to know
Today, let's talk about a key element in PCR experiments - consumables. As a frequent PCR visitor, it is necessary to understand the characteristics and terminology related to PCR consumables. There are many PCR consumables [...]