Cell therapy is rewriting the rules of modern medicine. Imagine a treatment that uses a patient’s own immune cells, reprogrammed in a lab to hunt down and destroy cancer—this is no longer science fiction but reality for thousands saved by CAR-T therapies.
Yet, behind these medical miracles lies an unsung hero: cell therapy manufacturing. Turning living cells into safe, consistent, and scalable treatments requires precision akin to building a microscopic “living pharmacy.”
In this article, we explore how scientists tackle this challenge and why the journey from lab bench to patient is as groundbreaking as the therapies themselves.
What is Cell Therapy?
How It Works
These therapies typically follow three steps:
Cell Sourcing: Cells are harvested (e.g., blood, bone marrow, or adipose tissue) and often genetically modified or activated.
Manufacturing: Cells are expanded, engineered, or primed in controlled environments to enhance therapeutic properties.
Delivery: Processed cells are reintroduced into the patient to target specific diseases.
Types and Applications
Immune Cell Therapies: CAR-T cells (genetically engineered to attack cancer) and TILs (tumor-infiltrating lymphocytes) are FDA-approved for blood cancers like leukemia and lymphoma.
Stem Cell Therapies: Mesenchymal stem cells (MSCs) reduce inflammation in autoimmune diseases, while induced pluripotent stem cells (iPSCs) aim to regenerate heart tissue or neurons.
Gene-Edited Cells: CRISPR-edited cells correct genetic defects, such as in sickle cell anemia.
Cell therapies aim to correct disease at its source by amplifying the body’s innate repair mechanisms—whether through reprogramming a patient’s own cells or introducing externally engineered counterparts. This approach shifts medicine from managing symptoms to restoring biological function. As research advances, the field is poised to redefine healthcare, offering not just treatments but long-term solutions tailored to the complexity of human biology.
Two Sides of the Coin: Autologous vs. Allogeneic Therapies
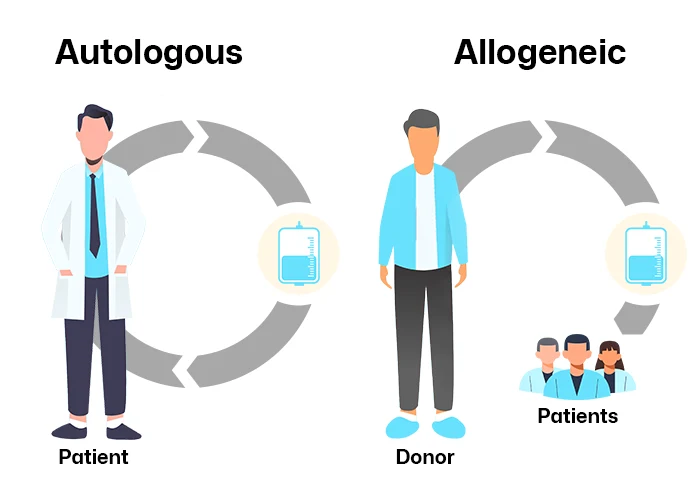
Autologous Therapies: The Ultimate Personalized Medicine
Autologous therapies begin with a patient’s own cells—often T cells for cancer immunotherapy or stem cells for regenerative treatments. These cells are harvested, engineered to target diseases (e.g., CAR-T modifications), expanded in bioreactors, and reinfused into the same patient.
Why Choose Autologous?
Immune Compatibility: Since the cells originate from the patient, they avoid immune rejection—a critical advantage for long-term efficacy.
Bespoke Targeting: Engineered cells can be tailored to the patient’s unique disease markers. For example, Yescarta® (axicabtagene ciloleucel), a CAR-T therapy for lymphoma, achieves 70% remission rates in relapsed patients by targeting CD19 proteins specific to their cancer cells.
The Catch:
Time-Critical Manufacturing: Processing a single batch takes 3–4 weeks, during which critically ill patients may deteriorate.
Sky-High Costs: Personalized production (often exceeding $500,000 per dose) stems from small-batch workflows and rigorous quality checks.
Cell Quality Variability: Chemotherapy-weakened patients may yield insufficient or dysfunctional cells, leading to manufacturing failures.
Real-World Impact: Autologous therapies dominate oncology, with six FDA-approved CAR-T therapies (e.g., Kymriah®, Breyanzi®) for blood cancers. However, their complexity limits accessibility—only 30–50 certified labs worldwide can produce them.
Allogeneic Therapies: The “Off-the-Shelf” Revolution
Allogeneic therapies aim to democratize access by using donor cells (e.g., umbilical cord blood, healthy T cells) mass-produced into frozen, ready-to-use doses. This “off-the-shelf” model eliminates the wait time and variability of patient-specific manufacturing.
Why Choose Allogeneic?
Scalability: A single donor batch can treat hundreds of patients, slashing costs to 50,000–100,000 per dose.
Immediate Availability: Pre-made doses enable urgent treatments, such as Allocord® for graft-versus-host disease (GVHD), administered within 48 hours.
Standardized Quality: Donor cells from healthy individuals ensure consistent potency, unlike autologous cells from frail patients
The Catch:
Immune Mismatch: Donor cells may attack the recipient’s tissues (graft-versus-host disease) or be rejected as “foreign.” To mitigate this, companies like Allogene Therapeutics use CRISPR to delete HLA genes—the molecular “ID tags” triggering immune responses.
Transient Efficacy: Allogeneic cells often survive only weeks, requiring repeated doses. For example, mesenchymal stem cell (MSC) therapies for Crohn’s disease typically need quarterly infusions.
Real-World Impact: Allogeneic therapies excel in non-cancer applications—Strimvelis® (for ADA-SCID) and Holoclar® (corneal repair) demonstrate long-term engraftment success. Over 60% of ongoing cell therapy trials now focus on allogeneic platforms, signaling an industry shift toward scalability.
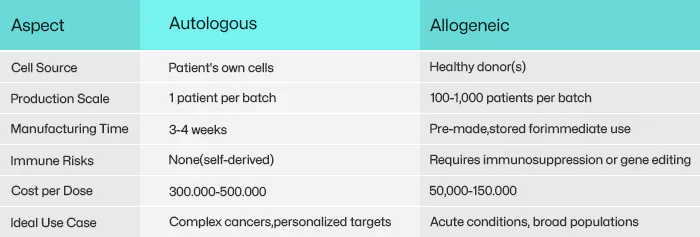
The Journey of Cell Therapy Manufacturing: From Patient to Pharmacy
1. Cell Sourcing: The Starting Line
The journey begins with cell collection, typically via blood draw (for immune cells) or tissue biopsy (for stem cells). Autologous therapies require meticulous handling to preserve cell viability during transport to manufacturing facilities. For example, CAR-T cells must reach labs within 24–48 hours at 4°C, while donor-derived allogeneic cells (e.g., umbilical cord blood) are banked in cryogenic freezers for on-demand use. But Patient cells from elderly or immunocompromised individuals often have low viability or contamination risks.
2. Processing and Engineering: The Customization Phase
In controlled cleanrooms, cells undergo activation, genetic modification, or reprogramming:
Viral Vectors: Lentiviruses deliver therapeutic genes (e.g., CAR constructs) into T cells.
CRISPR Editing: For allogeneic therapies, tools like CRISPR-Cas9 “knock out” immune-recognition genes (e.g., HLA class I) to prevent rejection.
Cell Expansion: Bioreactors act as “cell nurseries,” growing millions of cells into billions over 7–14 days.
Key Innovation: Closed-system bioreactors minimize human intervention, reducing contamination risks from 15% (open systems) to <1%.
3. Quality Control: The Gatekeeper
Every batch undergoes rigorous testing:
Identity: Flow cytometry confirms cell markers, e.g., CD19 CAR expression.
Purity: Tests detect contaminants like endotoxins or residual viral vectors.
Potency: Cells must kill 80% of target cancer cells in vitro.
The Bottleneck: QC accounts for 30% of production time and 40% of costs, as many tests require 7+ days (e.g., sterility assays).
4. Formulation and Delivery: The Final Mile
Cells are cryopreserved in liquid nitrogen (-196°C) or formulated for fresh infusion. Autologous therapies face a logistical race against time—viability drops 10% per day post-thaw. Allogeneic “off-the-shelf” products use stabilizers to extend shelf life (e.g., Mesoblast’s MSC therapies last 18 months at -80°C).
Even minor tools can make or break cell therapy production. During cryopreservation, for example, cells stored in pre-sterilized, leak-proof cryovials (like GenFollower’s sterile cryovials with dual O-ring seals) maintain 95% viability after thawing. In media preparation, filtered pipette tips prevent cross-contamination when handling viral vectors. Meanwhile, high-throughput ELISA plates (designed for rapid cytokine release assays) slash QC testing time by 30%, ensuring faster batch releases.
Why This Journey Matters
A single CAR-T batch involves 500+ manual steps and 50+ quality checkpoints. Yet, advancements like AI-driven process analytics and modular cleanrooms are slashing production times from weeks to days. Perhaps in the future, cell therapies will be as readily available as aspirin—driven by smarter manufacturing techniques.
4 Cell Therapy Manufacturing Approaches: From Lab Bench to Industrial Scale
1. Manual Open Systems: The Foundation of Personalized Medicine
Manual open systems represent the earliest and most flexible approach. In this method, technicians handle cells in open culture flasks or plates within biosafety cabinets (BSC). Every step—cell isolation, activation, genetic modification, and expansion—is performed manually, often using pipettes and centrifuges.
Strengths:
This approach thrives in research and early clinical trials, particularly for ultra-rare diseases requiring fully personalized therapies. For example, a patient-specific CAR-T therapy might begin with a technician extracting T cells from a blood sample, modifying them with a viral vector in an open system, and expanding them in small bioreactors. The low upfront cost (typically under $100,000 for basic equipment) makes it accessible for academic labs and startups.
Weaknesses:
However, open systems carry significant risks. Even in ISO 5-certified BSCs, human error or airborne contaminants can lead to batch failures. Industry surveys estimate contamination rates at 10–15%, often requiring costly repeat procedures. Additionally, labor intensity limits scalability—a single CAR-T batch may demand 20 technicians working in shifts over two weeks.
Current Applications:
Primarily used for autologous therapies in Phase I/II trials, where patient-specific customization outweighs inefficiencies.
2. Closed Automated Systems: Industrializing Cell Production
Closed automated systems emerged to address the pitfalls of manual methods. These self-contained platforms integrate cell processing steps—such as magnetic bead-based cell separation, electroporation for gene editing, and perfusion bioreactors for expansion—into single-use, sterile cartridges. Sensors monitor critical parameters like pH, dissolved oxygen, and glucose levels, reducing human intervention.
Strengths:
By eliminating open steps, contamination rates drop below 2%. Closed systems also standardize output, a necessity for commercial therapies like Yescarta® or Kymriah®, where regulators require batch-to-b consistency. A 2022 study showed that closed CAR-T production reduced labor costs by 50% and processing time from 14 days to 9 days.
Weaknesses:
High capital investment (500,000–2 million per system) and limited adaptability pose barriers. Once validated for a specific therapy (e.g., CD19 CAR-T), reconfiguring the system for a new target (e.g., BCMA CAR-T) requires costly revalidation. Additionally, single-use consumables generate substantial waste—a 500-liter bioreactor run can produce 200 kg of plastic waste.
Current Applications:
Dominates commercial autologous CAR-T production and allogeneic therapies with standardized protocols, such as mesenchymal stromal cell (MSC) therapies.
3. Modular Platforms: Bridging Flexibility and Scale
Modular manufacturing combines standalone devices (e.g., cell washers, bioreactors) into customizable workflows. Unlike closed systems, these platforms allow “mix-and-match” configurations—a clinic might use a modular setup to isolate NK cells with a magnetic separator, activate them in a static culture bag, and expand them in a rocking-motion bioreactor.
Strengths:
This approach balances scalability with adaptability. Contract development and manufacturing organizations (CDMOs) leverage modular systems to produce multiple therapies in shared facilities. For example, a CDMO might use the same bioreactor module for CAR-T and TCR therapies by swapping out culture media and sensors. Modular setups also reduce capital costs by 30–40% compared to fully closed systems.
Weaknesses:
Complexity rises with each added module. Operators must master disparate devices, and integrating data from multiple vendors’ equipment (e.g., bioreactor pH sensors vs. separator throughput rates) remains a hurdle. Furthermore, maintaining sterility across open transfers between modules demands rigorous protocols.
Current Applications:
Preferred for allogeneic therapies like NK cells or iPSC-derived products, where process optimization is ongoing and batch sizes vary widely.
4. All-in-One Systems: The Promise of Autonomous Manufacturing
All-in-one systems aim to revolutionize the field by consolidating all manufacturing steps into a single, fully automated platform. These “factory-in-a-box” solutions use artificial intelligence (AI) to control cell culture conditions, robotic arms for handling, and inline analytics for real-time quality control.
Strengths:
By minimizing human interaction, these systems achieve near-zero contamination risk. A 2023 pilot study demonstrated that an all-in-one system produced CAR-T cells with 99% viability and consistent potency across 50 batches. The compact design also enables decentralized manufacturing—hospitals could house these systems onsite, slashing logistics costs and time-to-patient.
Weaknesses:
Current platforms struggle with complex processes like viral vector integration or CRISPR editing, which still require manual intervention. Additionally, the lack of standardized interfaces between different vendors’ systems complicates integration. Early adopters report upfront costs exceeding $3 million per unit, though proponents argue long-term savings justify the investment.
Current Applications:
Experimental use in allogeneic “off-the-shelf” therapies, such as universal CAR-T cells or iPSC-derived cardiomyocytes, where automation can offset the complexity of large-scale differentiation.
Strategic Tradeoffs: Choosing the Right Approach
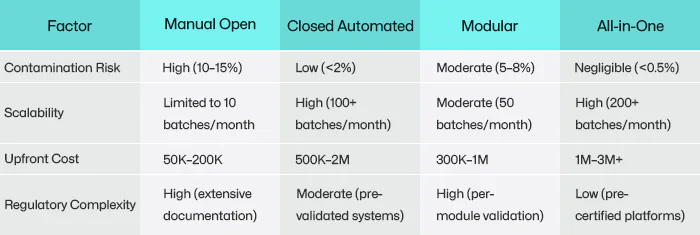
Data sources: 2022 ASGCT industry survey; contamination rates and costs are estimates—actual values vary by region and facility.
Advancing Cell Therapy Manufacturing Together
Cell therapy manufacturing faces dual challenges: scaling life-saving treatments while maintaining rigorous quality and cost control. Every component in this intricate process—from cell collection to cryopreservation—must function flawlessly.
GenFollower supports this mission through precision tools designed to address critical needs in cell therapy workflows:
Medical-grade cryogenic tubes: Made of pure polypropylene with silicone O-rings, these leak-proof vials withstand temperatures from -196°C to +121°C, ensuring secure storage in liquid nitrogen vapor phase. Features like color-coded caps and a curved bottom design streamline sample management.
Filtered pipette tips: Engineered to block 99.9% of aerosols during viral vector transfers, minimizing cross-contamination risks.
High-binding ELISA plates: Optimized to immobilize large biomolecules (≥50 kDa, e.g., antibodies) for accurate QC testing in vector production.
Medium-binding ELISA plates: Reduce non-specific interactions for low-molecular-weight targets (<50 kDa), lowering background noise in cytokine release assays.
By focusing on reliability across consumables—from PCR tubes to sterile culture dishes—GenFollower helps researchers mitigate contamination risks and accelerate timelines.
The future of cell therapy hinges not only on breakthroughs in science but also on perfecting the tools that bridge discovery and delivery. Together, we can transform manufacturing challenges into milestones for patient impact.
PCR Tubes vs. Strips vs. Plates: How to Choose the Right Format for Experiment’s Throughput & Accuracy
Have you ever faced inconsistent PCR results due to poor sealing, or wasted hours manually handling single tubes in high-throughput workflows? These challenges not only delay your experiments but can also compromise data accuracy. A [...]
The Ultimate Guide to Syringe Filters
Filtration plays a critical role in ensuring the purity and reliability of solutions across industries, from pharmaceuticals to environmental testing. Among the most widely used filtration tools are 0.22µm and 0.45µm filters, known for [...]
HPLC Troubleshooting Guide
High-Performance Liquid Chromatography (HPLC) is a cornerstone analytical technique widely used in chemistry, biochemistry, and pharmaceuticals for separating, identifying, and quantifying components in complex mixtures. Its precision and versatility make it indispensable for quality [...]